Actualidad
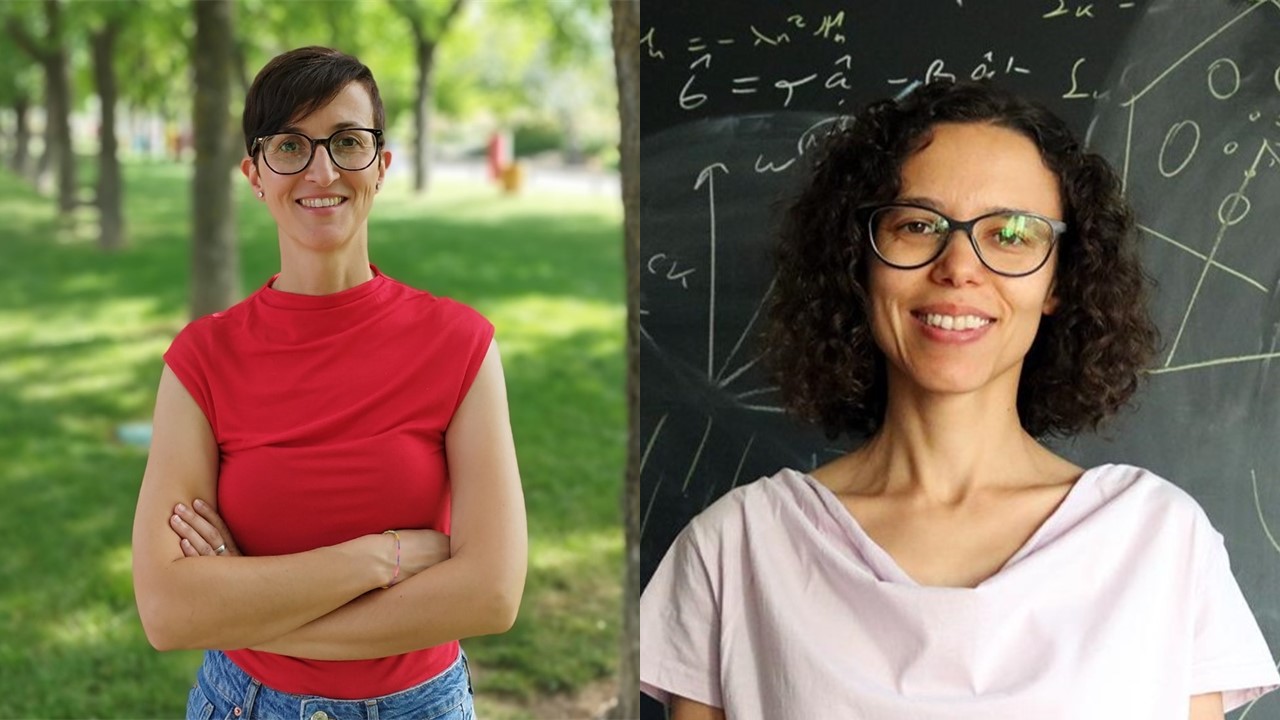
Fátima Aparicio Hernández y Paloma Arroyo Huidobro reciben el Premio Jóvenes Investigadoras de la Universidad Autónoma de Madrid (UAM) en su edición 2024
La concesión de estos Premios busca distinguir a jóvenes investigadores e investigadoras cuyo trabajo haya contribuido de forma relevante al desarrollo científico en la UAM